NB: This article is part of the Bioenergetic Blueprint series. The series is meant to be read in order. You can find its table of contents right here.
Around 400 BC, pre-Socratic thinker Democritus proposed the avant-garde idea that all matter is made up of tiny, “indivisible” particles known as atomos (Greek word for “uncuttable”). More than twenty-two centuries later, he was proven right.
I’m sure you already know more or less what an atom is and how it’s structured from high-school chemistry, but given that we are going to discuss orbitals and redox reactions in some detail after this, I wanted to provide a quick introduction to the humble atom and its main characteristics beforehand.
So, let’s get right into it.
Subatomic Particles
Atoms are made of three main subatomic particles:
Protons
Protons are positively charged particles.
They live in the nucleus of an atom.
The number of protons of an element (AKA the atomic number) defines the element. When the number of protons changes, the element in question changes with it.
For instance:
Carbon (C) has 6 protons. We can verify this because the atomic number is written on the periodic table, above the element. If it had 7 protons, it would be nitrogen (N).
Neutrons
Neutrons are electrically neutral particles.
They also live in the nucleus of an atom, alongside protons.
The number of neutrons of an element doesn’t define the element: it determines which variation of said element the atom is. These variations are called isotopes.
For instance:
“Basic” carbon has 6 protons and 6 neutrons. We call this isotope “Carbon 12”, where the 12 is what’s known as the mass number, which corresponds to the sum of protons and neutrons.
However, there exist other carbon isotopes, such as Carbon 13. This isotope has the same number of protons (because it’s still carbon) but a different amount of neutrons. Therefore, just by their name and mass number, we can know Carbon 13 has 6 protons and 7 neutrons; Carbon 14, has 6 protons and 8 neutrons, etc.
The specific role of neutrons seems a bit mysterious, but one of their most crucial functions might simply be providing enough cushion between protons, helping them stick together and keeping the nucleus stable.
Electrons
Electrons are negatively charged particles.
Electrons are approximately 2,000 times tinier than protons and neutrons.
Unlike protons and neutrons, they don’t live in the nucleus of an atom; instead, they live in what are called orbitals.
Electrons are far more interesting than protons and neutrons, so we’ll cover a couple of their properties below, and dedicate the next couple of articles to discussing how they interact with one another.
Electron Characteristics
Ions, Cations, Anions
Because protons are positively charged, and electrons negatively charged, if an atom has the same number of both, their charges will cancel, and we will say the atom has no charge.
If, however, an atom does not have the same number of protons as electrons, it will have an overall positive or negative charge, and we will call this atom an ion. So, an ion is nothing more than an atom/molecule whose total charge is different from zero. You can easily spot ions because their charge is indicated in the top-right corner of the element.
Ions are subdivided into two types:
When an ion has an overall positive charge, it means the positive charge from its protons is greater than the negative charge from its electrons. We call this positive ion a cation.
For instance: Mg+2 is a magnesium cation because it has a positive charge of 2, indicating it has 2 more protons than it does electrons.
When an ion has an overall negative charge, it means the positive charge from its protons is less than the negative charge from its electrons. We call this negative ion an anion.
For instance: F- is a fluorine anion because it has a negative charge of 1, indicating it has 1 less proton than it does electrons.
Energy Levels
Not all electrons are equal. Some are said to have higher energy levels than others. Energy levels are often represented by what we call electron shells, which are layers surrounding the nucleus of an atom. These shells are designated by their principal quantum number, starting from the closest to the nucleus (n = 1) and moving outward as the energy level increases.
We can interpret energy levels in terms of the electron’s distance from the nucleus and its inherent negative charge:
The farther away an electron is from its nucleus, the higher in energy it is said to be. Because electrons have an inherent negative charge, an electron with higher energy can be thought of as being less negative. Think of it like -2 being a higher number than -4.
The closer an electron is to its nucleus, the lower in energy it is said to be. Because electrons have an inherent negative charge, an electron with lower energy can be thought of as being more negative. Following the numerical analogy: -4 is a lower number than -2.
You can also think of this in terms of potential energy, which we touched on in this article. As electrons get pulled farther and farther away from the nucleus, their energy increases because their potential energy increases. It’s the subatomic equivalent to pulling the string of a bow: the farther they get from the nucleus, the more they want to snap back towards it and reach a stabler, lower energy state.
This farther-closer dynamic makes sense if you recall that an atom’s nucleus is positively charged and that electrons are negatively charged; and because their charges are opposite, they are attracted to one another, like magnets. Therefore:
Electrons with lower energy levels are more strongly attracted to the nucleus and thus reside closer to it.
Electrons with higher energy levels are less strongly attracted to the nucleus, resulting in them being farther from it.
When we think of electrons in this way, we can begin to group them within said “shells” or “layers” that build out away from the nucleus, depending on their electrical charge, as Bohr’s famous diagram model captures.
Valence Electrons
It is precisely an atom’s constantly changing, but ultimately stable outermost electron shell (i.e., highest energy electrons) that is involved in reactions with other atoms, because it is the shell least attracted to its atom's nucleus. We call this shell the valence shell.
The number of valence electrons in an atom dictates its chemical behavior. Atoms in the same column of the periodic table share the same number of valence electrons, resulting in similar reactive characteristics. This trait is notably apparent in groups like the inert noble gases, which possess a full valence shell and exhibit minimal reactivity. We’ll discuss the example of neon in the next article.
In their quest to emulate the stability of noble gases, many elements follow what’s known as the octet rule: striving to house eight electrons in their valence shell (not in total). We can see how many electrons are needed to fulfill this rule just by looking at a slice of the periodic table.
On the rightmost column, we have noble gases, which are most unreactive, precisely because they already have 8 valence electrons, so they don’t need more. Moving left, we get elements like fluorine (F) which have 7 electrons in its valence shell, so it needs 1 more to achieve a full octet. Then we have oxygen (O), which has 6 electrons in its valence shell and thus needs 2 to fulfill the octet. Nitrogen (N) has 5, so it needs 3. Carbon has 4, so it needs 4. This rule is far from perfect and has many exceptions, but it’ll make do for our purposes here.1
In order to complete their octets, atoms engage in two types of bonds:
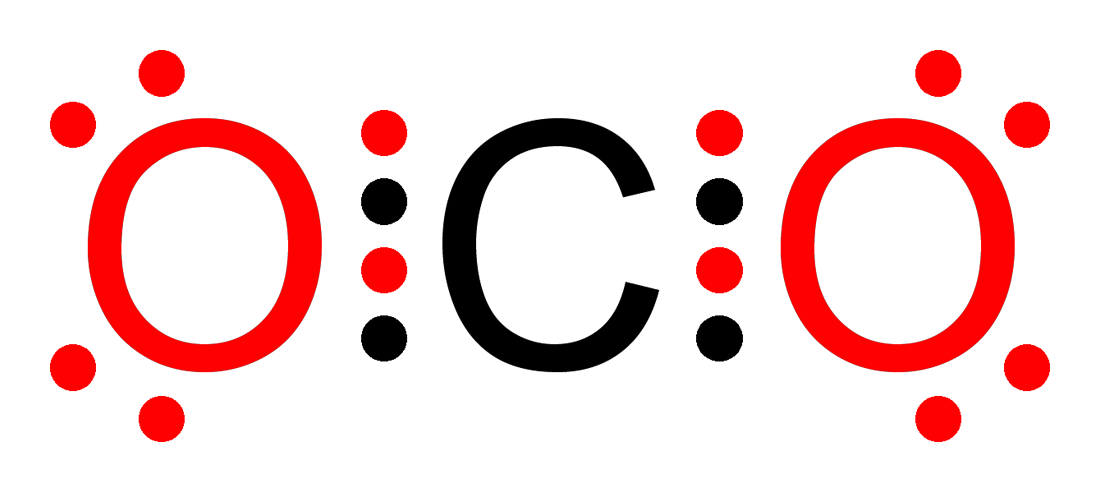
In covalent bonds, atoms share electrons. This sharing of electrons is fundamental in constructing many organic compounds. A quintessential example is water (H₂O), where each hydrogen atom shares one of its electrons with the oxygen atom. Through this sharing, the oxygen atom completes its octet. Another example would be carbon dioxide (CO2) as pictured above.
Alternatively, atoms can completely transfer electrons to or from another atom. This type of bond is known as an ionic bond because it creates ions in the process. Sodium chloride (NaCl) is a classic example. Sodium, with one electron in its outer shell, donates one electron to chlorine, which has seven valence electrons. Sodium becomes a positively charged ion (Na⁺), and chlorine becomes a negatively charged ion (Cl⁻). Because both ions are now oppositely charged, they are attracted to one another, and so they form an ionic bond.
We will discuss this type of bonds and what guides them in much more detail when we talk about redox reactions.
Electronegativity
Electronegativity is a measure of an atom’s ability to attract and hold onto electrons within a chemical bond. You can think of it as an atom’s “greediness” for electrons.
If you imagine two atoms forming a bond as individuals engaged in a tug-of-war over electrons, electronegativity would be a measure of how strongly each atom “tugs” on the (electron) rope. Atoms with higher electronegativity exert a greater pull, drawing the electrons closer; atoms with lower electronegativity are weak and pathetic.
The disparity in electronegativity between bonding atoms dictates the nature of their bond:
Low differences in electronegativity often result in covalent bonds, because both atoms share the electrons more or less equally. Here, each atom satisfies its electron requirements without overshadowing the other, resulting in a stable (“happy”) bond. The resulting compound is described as nonpolar because there is no significant charge difference across its structure—both atoms maintain a harmonious balance.
Medium differences in electronegativity often result in covalent bonds too, but ones which are polar. Here, electrons are shared, but unequally: one atom exerts a stronger pull on the shared electrons, leading to a scenario where electrons linger more around one atom than the other. The resulting compound is termed polar because it has partial positive and negative sides, akin to the poles of a magnet.
High differences in electronegativity often result in ionic bonds, where one of the atoms outright “steals” the electrons from the other. This transforms the atoms into ions: the electron-receiver becomes negatively charged, while the electron-giver becomes positively charged.
We can actually measure the electronegativity of elements using the Pauling scale (named after Linus Pauling):
On the lower end of the spectrum, elements like francium and cesium have electronegativities around 0.7, reflecting their propensity to lose electrons and thus form cations. This low electronegativity is a signature trait of metals.
Around the middle of the scale, we find carbon, with an electronegativity of about 2.5. Just by this, we can see why carbon is the organic element par excellence: its medium electronegativity makes it versatile and able to form all types of bonds.
At the higher end of the scale, we find elements like oxygen, with an electronegativity of approximately 3.5, AKA high affinity for electrons. As we will see, in our bodies, oxygen acts as the ultimate “electron acceptor”, so it makes sense for it to be very electronegative.
We mentioned in previous articles that “life has successfully evolved (and continues to evolve) because, at a fundamental level, matter has managed to (1) interpose itself over the flow of energy and (2) elaborate itself by exploiting its current.” We can now begin to understand that, for aerobic organisms like ourselves, this flow of energy and its current is ultimately composed of electrons making their way toward oxygen.
Conclusion
Atoms are the fibers that weave the grand tapestry of nature. As such, their electron configuration is absolutely crucial to our understanding of energy metabolism because they largely determine how they interact with each other. In the next article, we’ll start taking a better look at how electrons behave in single atoms, so we can later understand how electrons from different atoms actually form bonds with each other.
Upwards,
Yago
Want more articles like this one?
Leave a comment, a like, and share it with your loved ones!
Premium Courses
Modern Detox: Cleanse yourself from the modern world
Nurturing Nature: Cultivate yourself with timeless habits
Free Resources
Impero Wiki: The resource hub for aspiring Renaissance Men
The following exceptions can break the octet rule:
Third row of elements and lower, because they can have a d orbital subshell and thus house more electrons (more on orbitals in the next articles).
Hydrogen (H) can only have 2 valence electrons; Beryllium (Be), 4; Boron (B) and aluminum (Al), 6.
Molecules with odd numbers of electrons, as they cannot evenly distribute electrons to achieve a complete octet.
Takes me back to doing my chemistry & physics at school!
Might have to go and dig out a couple of my old work books...
This is an OUTSTANDING overview -- THANK you !!